Information
Authors: Robert Ivkov, Sally J. DeNardo, Wolfgang Daum, Allan R. Foreman, Robert C. Goldstein, Valentin S. Nemkov, and Gerald L. DeNardo
Conference/Publication: Journal of Clinical Cancer Research 11 (19 suppl)
Topic: Cancer Treatment
Abstract
Methods - Thirty-seven female BALB/c athymic nude mice (5-8 weeks) were exposed to an AMF with frequency of 153 kHz, and amplitude (400-1,300 Oe), duration (1-20 minutes ), duty (15-100%), and pulse ON time (2-1,200 seconds). Mice were placed in a water-cooled four-turn helical induction coil. Two additional mice, used as controls, were placed in the coil but received no AMF exposure. Tissue and core temperatures as the response were measured in situ and recorded at 1-second intervals.
Results - No adverse effects were observed for AMF amplitudes of V700 Oe, even at continuous power application (100% duty) for up to 20 minutes. Mice exposed to AMF amplitudes in excess of 950 Oe experienced morbidity and injury when the duty exceeded 50%.
Conclusion -High-amplitude AMF (up to 1,300 Oe) was well tolerated provided the duty was adjusted to dissipate heat. Results presented suggest that further tissue temperature regulation can be achieved with suitable variations of pulse width for a given amplitude and duty combination. These results suggest that it is possible to apply high-amplitude AMF (>500Oe) with pulsing for a time sufficient to treat cancer tissue in which magnetic nanoparticles have been embedded.
Introduction
When living tissues are heated to temperatures between 42°C and 46°C the result is cellular inactivation in a dose-dependent manner (1, 2). Some success has been achieved in the development of cancer treatments based on this classic hyperthermia response (3 – 6). Tissues heated to above 46°C undergo extensive necrosis known as thermoablation. The promise of thermoablation has been realized for some local and regional diseases (7, 8).
The concept of thermoablative cancer therapy requires that a controlled amount of heat be focused in the tumor area without excessively heating the intervening tissues. A variety of strategies have been developed that use the alternating magnetic field (AMF) component of electromagnetic fields in the radiofrequency spectrum to localize and concentrate ablative heat for cancer treatment by either directly heating the tissue or activating a susceptor material. These techniques take advantage of local heat production through either (a) induction heating of a material embedded within the tissue, or (b) direct tissue heating caused by the interaction of radio-frequency with the tissue, or (c) both.
Induction heating of magnetic (susceptor) materials embedded in cancer tissue, also called hysteresis heating, results from the interaction of the magnetic moment of the susceptor material with the AMF. On the other hand, interaction of AMF with tissue can produce heat directly, as with any electrically conductive material. The mechanism that dominates this type of heating results from the production of (electric) eddy currents producing heat that scales as where SAR is the tissue-specific absorption rate, measured as W/g tissue, r is the radius of exposed region, an f and H are the AMF frequency and amplitude, respectively. Thus, the energy source for the susceptor material is also the heat source for nonspecific heating of intervening tissue.
An approach using AMF to heat magnetic nanoparticles embedded in cancer tissue has the potential to provide selective heating to the microenvironments of cells containing the excited particles (9 – 11). The degree to which this approach is practical depends upon (a) the ability to systemically deliver nanoparticles to the cancer in sufficient concentrations to achieve thermoablation when AMF is applied and (b) a combination of magnetic nanoparticle and AMF characteristics that result in extremely rapid heating of the specific cancer microenvironment while maintaining temperatures tolerable to normal tissues.
AMF combinations that are ideal for rapid particle heating (i.e., high amplitude and long durations) can deposit power to tissues challenging mammalian thermoregulatory mechanisms. These mechanisms maintain the body temperature within a prescribed temperature range under conditions in which the thermal load on the body may vary. Exposure to high-amplitude AMF in the radiofrequency range provides a unique exception to energy flows normally encountered by mammals (12). Thus, it is beneficial to explore AMF variables that selectively induce eddy current heating without significantly reducing potential for particle heating.
This report describes the safety implications of a new technique to treat cancer that exploits the combination of magnetic nanoparticles localized in cancer using specific antibodies (bioprobes) and an AMF device that can be switched on and off to heat the targeted nanoparticles thereby inducing heat at the cancer sufficient to destroy the cancer cells. This novel treatment is unique, because the heat is focused on the cancer cells by virtue of the localization of the nanoparticles so that when the magnetic field is applied, the bioprobes heat and destroy the cancer cells but provide relative sparing of the healthy tissues. This study was intended to define the implications of the nonspecific heating incident to the AMF.
The limits of safe application of confined, pulsed, high-amplitude (400-1,300 Oe) alternating magnetic fields with frequency of 150 kHz in vivo in a mouse model were explored in anticipation of a future study intended to treat cancer using submilligram quantities of bioprobes embedded in cancer tissue. An AMF inductor was built to confine high-amplitude magnetic fields to a 1-cm wide band of the interior of a 35-mm internal diameter induction coil. Mice were subjected to varying combinations of AMF by adjusting amplitude, duty, pulse ON/OFF time combinations, and total duration of exposure.
High-amplitude AMF was well tolerated even at 1,300 Oe for up to 20-minute exposures provided the duty and ON/OFF combinations were adjusted to allow sufficient OFF time to dissipate heat. At lower field amplitudes of ≤700 Oe, no adverse effects were observed even at continuous power application (100% duty) for up to 20 minutes.
Materials and Methods
Mice
A total of 39 female BALB/c nu/nu mice (Harlan Sprague-Dawley, Frederick, MD), 5 to 8 weeks old and weighing 20.0 to 29.2 g (mean, 26.3 g), were maintained according to University of California guidelines on a normal diet, ad libitum.
Each mouse was anesthetized by injecting 0.02 mL i.p. per gram body weight of a solution prepared by dissolving 0.5 g of 2,2,2-tribromoethanol in 1.0 mL warm tert-amyl alcohol then diluting the solution with 40 mL distilled water and filtering through a 0.2-µm filter. Anesthesia was determined by lack of reflexive response when a hind paw was lightly compressed.
After the mouse was anesthetized, four fiber optical temperature probes (FISO, Inc., Quebec, Canada) were placed. One was inserted s.c. proximal to the lower spine (spine) by inserting a 16-gauge x 1.5 in. hypodermic needle at the base of the tail and threading the fiber optical probe through the needle under the skin. This procedure was repeated for a second probe placed s.c. in the flank (flank). A third probe was taped onto the skin of a hind limb (skin) using wound dressing, and a fourth probe was inserted one cm into the rectum (rectal). After the probes were in place, the mouse was wrapped lightly in absorbent paper and inserted into a 50-mL centrifuge tube with the bottom removed. This tube with the mouse was inserted into the felt-lined AMF coil, so that the intended abdominal tumor location of each mouse was positioned in the 1-cm high-amplitude region of the induction coil. Once the mouse was in place and the variables programmed into the controls, the AMF generator was turned on. The magnetic field and time variables sampled in this study are summarized in Table 1.
After exposure, each mouse was left in the coil until the core (rectal) temperature began to decrease and all probes were removed. The mouse was removed from the coil and centrifuge tube and placed on a warm recovery pad on its back. When the righting reflex returned, the mouse was returned to its cage.
The mice were observed for 48 hours for signs of morbidity. Mice that died (total of five, cf. Table 1) during that period were necropsied. One mouse that showed no sign of injury was randomly selected and euthanized for comparison (Table 1).
Temperatures were recorded at 1-second intervals for each probe, beginning after each mouse was positioned in the coil and 30 seconds before AMF exposure. All mice placed in the coil exhibited decreasing temperature (hypothermia) before initiation of AMF exposure, likely due to the combination of anesthesia (12) and the 14°C inductor coil. Initial temperatures varied from 26°C to 32°C. Two mice that received no AMF exposure but were otherwise prepared in the same manner were placed in the coil to provide control data to compensate for this temperature change. For each mouse receiving AMF, the initial temperature was subtracted from temperature at time t = 1, 10, 20 minutes and corrected using averaged control data. A summary of the corrected temperatures due to AMF, ∆Tcorr, is provided in Table 2.
Alternating magnetic field system
A system was designed and built to provide high-amplitude AMF in an ~1-cm-wide band to the lower abdomen of a mouse. The lower abdomen was the intended site of future cancer xenograft, and by confining exposure to high-amplitude AMF to this region, exposure of tissue outside the intended area was minimized. The system consisted of three main components: (a) the induction coil, or inductor (Fig. 1A and B); (b) a capacitance network that, when combined with the inductor, forms a resonant circuit; and (c) the power supply.
Inductor The inductor was designed using Flux 2D (Magsoft, Inc., Troy, NY) finite element analysis software. The induction coil was manufactured from square cross-sectional, oxygen-free, and high-conductivity copper tubing. The length of the induction coil was 40 mm with an internal diameter of 36 mm, which enabled insertion of a 50-mL conical centrifuge tube containing a mouse and allowing for 2.5 mm of thermal insulation around the circumference of the 50-mL tube.
A low-reluctance flux-concentrating ring made of Fluxtrol 50 (Centre for Induction Technology, Auburn Hills, MI) was added to the end of the solenoid coil where the mouse was inserted to divert magnetic flux away from areas not intended to receive high-amplitude AMF exposure. A low-reluctance magnetic return path was also added to one fourth of the outer diameter of the coil. These components provided a preferential path for the magnetic flux that minimized AMF exposure to much of the mouse body and directed the flux in the interior of the solenoid producing a 1-cm band of high-amplitude AMF in the ventral region, the intended tumor location.
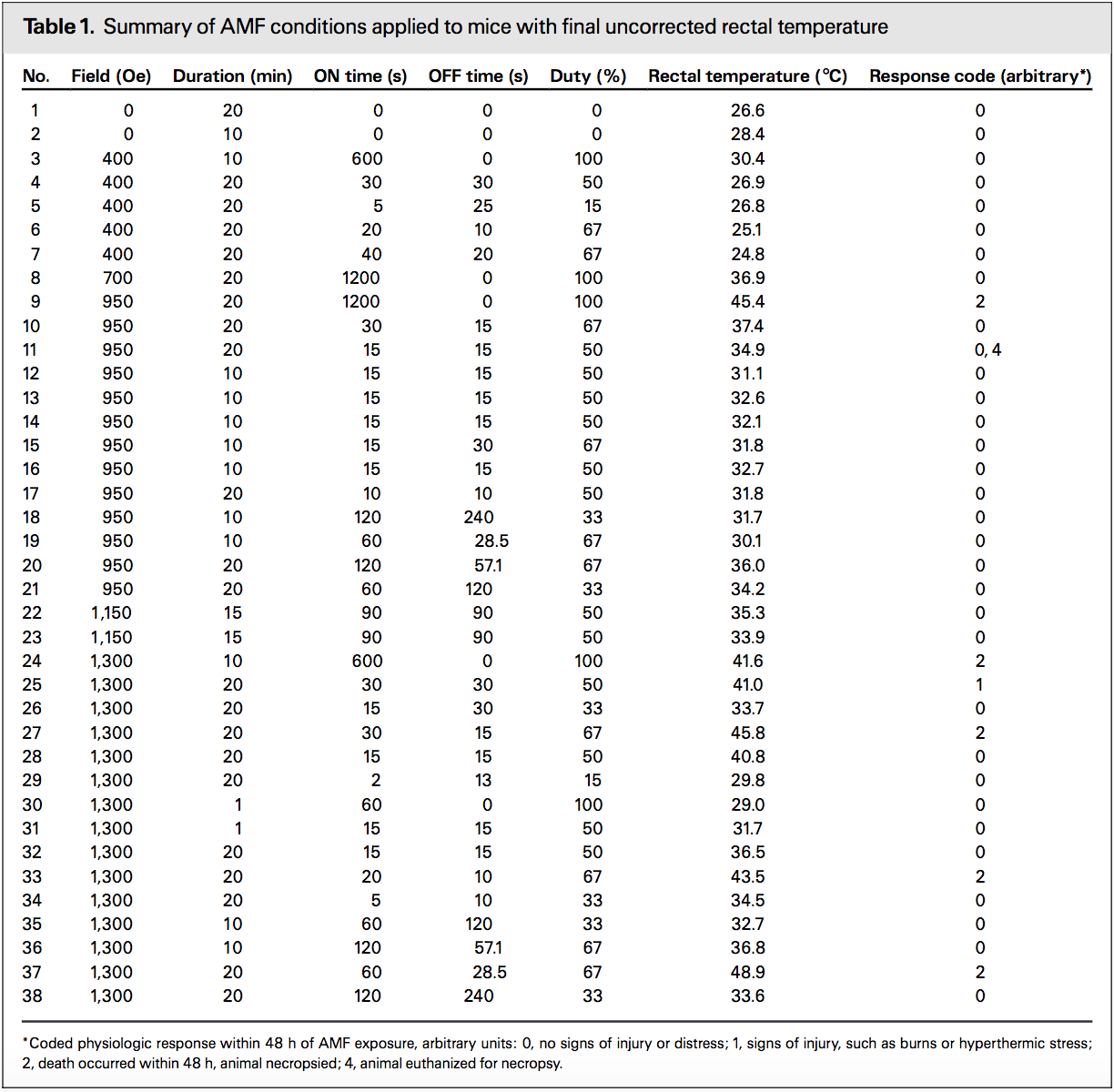
Measurements of the AMF flux with a current probe confirmed that if the rear third of the mouse was inserted into the induction coil, a 1-cm region of the abdomen could receive as much as 1,380 Oe, whereas tissue outside the concentrating ring (over 50% of the mouse) would not be exposed to >200 Oe. The field amplitude within the 1-cm band was measured before each set of trials and for each generator power setting used. It is the amplitude within this region that is reported as AMF amplitude.
During operation, the induction coil itself heats because it is a conductor carrying a high current load, particularly at high amplitude and high duty. To compensate, the inductor was cooled using a closed-loop circulating water system maintained at 14 ± 2°C during operation.
Capacitance network and power supply. The capacitance network was built into the power supply and was adjusted for stable oscillation at 153 ± 0.5 kHz. The power supply was a 25-kW (0-25 kW) generator manufactured by PPECO (Watsonville, CA). A pulse-timer circuit manufactured by Giltron, Inc. (Medfield, MA) was installed allowing 0.5- to 9,999-second pulses at any duty (0-100%), defined as:
Experimental design and data analysis
Statistical design and data analysis. For both study design and data analysis, we used a statistical approach that did not require a priori knowledge of the effects and interactions of input variables and responses (i.e., AMF conditions and in vivo temperature, respectively). A total of five input variables were simultaneously varied: (a) field amplitude (Oe), (b) total duration of exposure (minutes), (c) duty (%), (d) pulse ON time (seconds), and (e) pulse OFF time (seconds). The first three of these variables were sufficient to define the total AMF exposure. The latter two variables have an influence upon the unsteady state thermal evolution, particularly with respect to physiologic and environmental heat dissipation.
Trial design was done using Stat-Expert software (Stat-Ease, Inc., Minneapolis, MN) using both D-optimal and Box-Behnken designs to select trial conditions and numbers of mice that minimized both numbers of mice and morbidity.
Mice were divided into two groups, defined by short ON pulses (0-30 seconds) with field amplitudes of 400, 950, and 1,300 Oe and long ON pulses (60-240 seconds) with field amplitudes of 700, 1,150, and 1,300 combinations. In the second group of mice, factors selected from those used in the first group were repeated to maintain statistical continuity, allowing us to combine data from both groups for analysis. Also in the first group of mice, some mice were exposed to continuous power AMF (100% duty) for up to 1,200 seconds.
Temperature was measured as the response to AMF exposure. For analysis, a mathematical model of the form was fit to the temperature data using the method of least squares, where Y is the response, or temperature, and X1, X2, ... represent the factors duty, field amplitude, and duration. Temperature response to the combination of field amplitude, duration, and duty was analyzed. The effects of each term were determined using standard ANOVA techniques. Analysis and graphical representation of the data were done using Stat-Expert software (Stat-Ease). Physiologic responses were also noted; however, a systematic study of these was not undertaken.
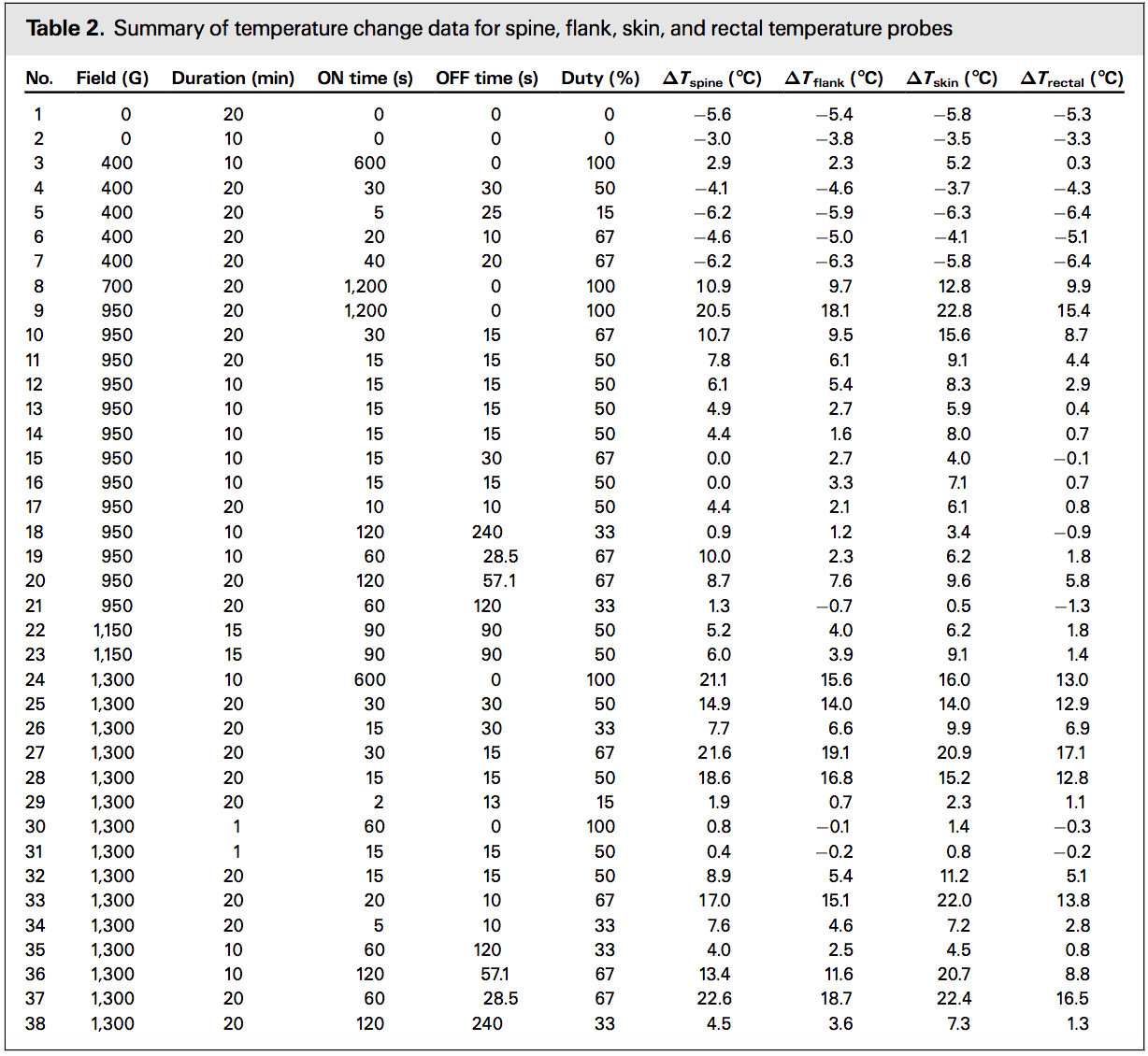
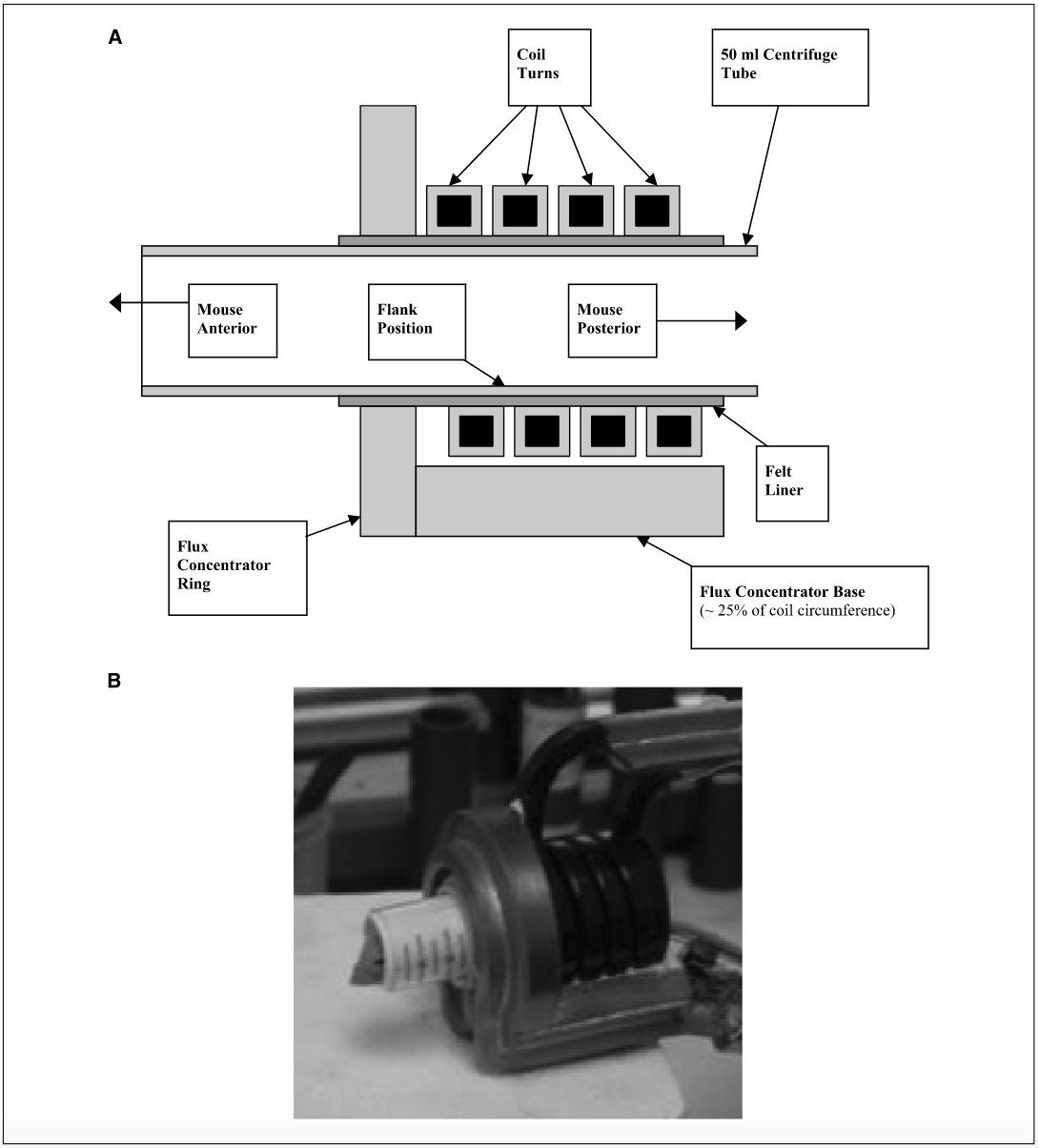
Further analysis of the data including ON/OFF time combinations was undertaken to ensure the validity of the model, because all factors studied are not mutually independent, or orthogonal. Duty is an explicit ratio of ON and OFF times (Eq. B) and should therefore be separated during analysis. Conversely, duty is a measure of average power (or average ‘‘dose’’), and for comparisons among specific ON/OFF combinations, it is natural to consider the effects of pulse width by choosing a combination of duty-ON time or duty-OFF time.
Therefore, two separate analyses of the data were done in addition to that using the combination of field amplitude, duration, and duty. Specifically, the combination of field amplitude, duration, ON time, and OFF time was analyzed to determine effects of pulse width on temperature, independent of duty. These results were compared against an analysis using the combination of field amplitude, duration, duty, and ON time.
Results
Table 1 contains a summary of AMF exposure conditions and final uncorrected rectal temperatures for the mice, whereas Table 2 provides a summary of ∆Tcorr for all temperature probes. Mice that experienced final rectal temperatures approaching or exceeding 42°C consistently died, with one exception, for which a rectal temperature of 47.3°C was recorded (data not shown). This datum was excluded from analysis by standard statistical criteria.
Mice exposed to field amplitudes in excess of 950 Oe and at duties >50% experienced consistent morbidity and injury. In these mice, maximum uncorrected rectal temperature approached or exceeded 42°C (Table 1), and ∆Tcorr was observed to be greater than ~12°C (Table 2). The average start temperature for all mice was 30.5 ± 0.3°C for the rectum, 29.0 ± 0.2°C for the skin, 30.7 ± 0.3°C for the flank, and 28.8 ± 0.3°C for the spine.
When injury or death occurred, necropsies of mice that died within 48 hours after AMF exposure (Table 1) showed consistent findings. The skin under the first inductor turn was noticeably red. Sometimes the exterior of the hind legs showed petechiae. Inside the abdomen, intestine that had been within the first inductor turn closest to the abdominal wall were red but not hemorrhagic. The cecum sometimes showed petechiae. Intestine deeper in the abdomen were pale and sometimes blanched. Lungs were red but not hemorrhagic. By comparison, necropsy results were normal in all respects of the one mouse randomly selected from the group showing no sign of injury (Table 1).
Observed temperature changes (i.e., ∆T) with time as a response to AMF amplitude of 1,300 Oe and 33% duty for temperature probes placed on the skin and in the rectum are shown in Fig. 2A and B, respectively. These temperatures are not corrected against controls and represent ON/OFF combinations of 5/10, 15/30, and 120/240 seconds corresponding, respectively, to mouse numbers 34, 26, and 38 in Tables 1 and 2.
Fitting the model equation (Eq. C) to corrected temperatures (∆Tcorr) with field, duty, and duration as factors produced statistically significant (P ‹ 0.05) results for spine, flank, skin, and rectal temperature responses for cubic (total sum of exponents, ≤3) combinations of the factors and their interactions. Contributions from higher order terms (i.e., exponent >3) were not significant nor was a model that included these terms. Other statistical measures of significance such as R^2 values, analysis of residuals and outliers, lack of fit tests, and tests of adequate precision (signal to noise) confirm the validity of the model. Thus, analysis and predictions within the variable space were validated.
The resulting model equation was used to generate a series of two-dimensional contour plots on which contour lines represent isotherms of response (constant ∆Tcorr). A sample of contour plots for 20-minute exposure showing the relationship between duty and field amplitude for spine, flank, skin, and rectal ∆Tcorr is displayed in Fig. 3. The contour plots show that the variable space displayed was well represented by experiment. Note, however, that the circles represent conditions at which data were taken and do not indicate the measured ∆Tcorr nor do they indicate by their placement a relationship with the predicted ∆Tcorr.
The effects of duration of exposure on rectal ∆Tcorr are compared in Fig. 4A to C for 5-, 10-, and 20-minute exposures at 400 Oe (Fig. 4A), 700 Oe (Fig. 4B), and 1,300 Oe (Fig. 4C). In general, increasing ∆Tcorr accompanies increasing duration of exposure and duty. However, at low duties and low-amplitude AMF (Fig. 4A-B), long durations in the coil do not produce substantial temperature increases and may even accompany a decrease of rectal ∆Tcorr. This indicates that AMF heating is insufficient to overcome heat loss. With AMF amplitudes of >700 Oe, sufficient eddy current heating occurs at duties >50%, eventually becoming acute and leading to adverse events. However, at the highest amplitudes (~1,300 Oe), duty has a profound effect on AMF-induced eddy current heating (cf. Fig. 4C).
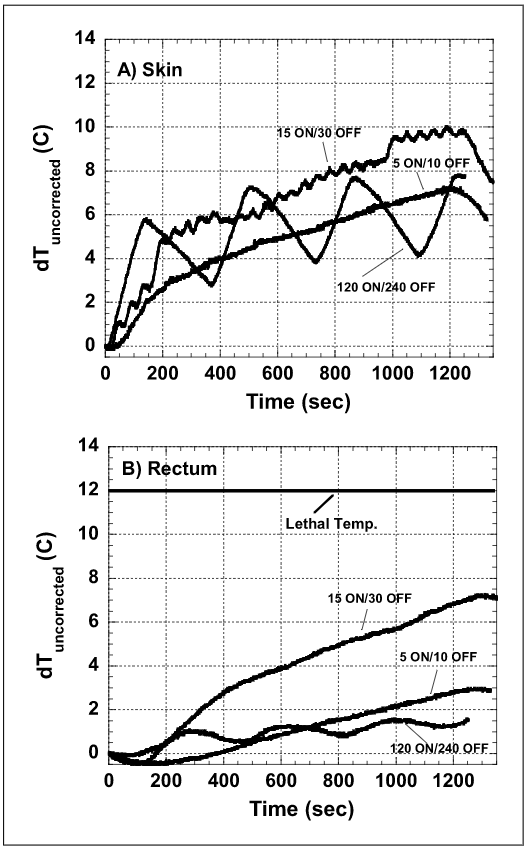
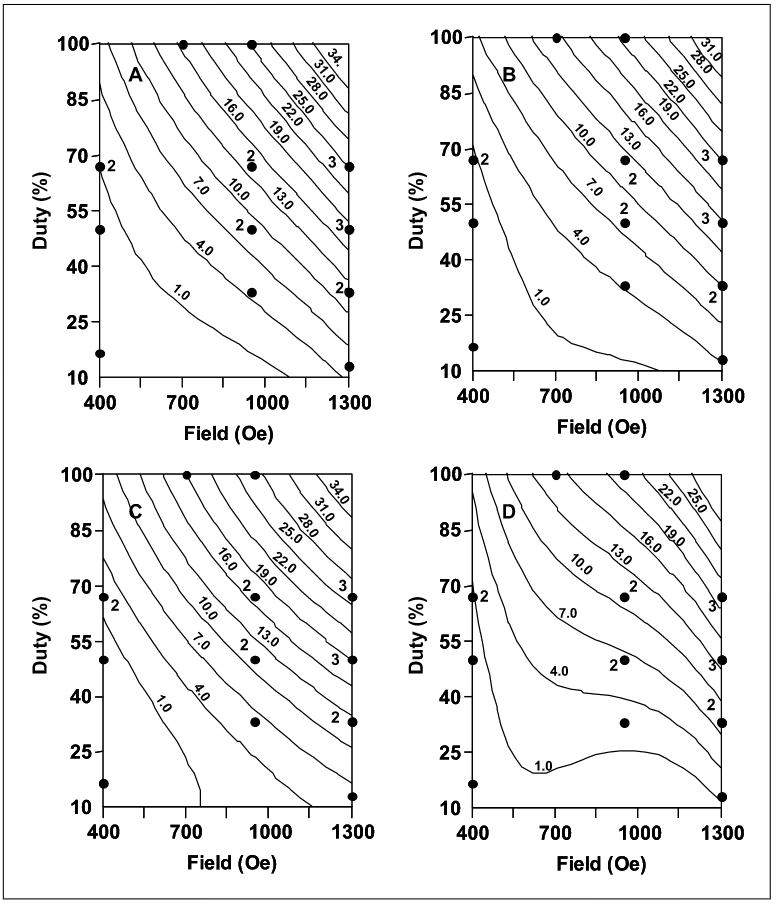
A similar analysis was done for field amplitude, duration, ON time, and OFF time. These factors produced statistically significant (P ‹ 0.05) ∆Tcorr responses for only linear combinations of the factors and their interactions. Unlike the previous analysis, not all factors were significant in the statistical analysis. Only field, field-duration, and duration-OFF time combinations were statistically significant factors in the response. Other statistical measures confirmed the validity of the model; however, by comparison with the first model, the SEs of the estimated coefficients were larger. This perhaps indicates that a greater number of data points are required due to the additional factor. Still, the model provides an adequate signal and is valid for predicting ∆Tcorr within the design space.
In a final analysis the effects of field amplitude, duration, duty, and ON time on ∆Tcorr were compared. Statistical measures of validity were similar to those obtained for the field-duration-ON-OFF analysis and even slightly better. Both models produced qualitatively similar predictions for ∆Tcorr when analogous combinations of the respective factors were used.
Because four factors were used to analyze the effect of pulse width (i.e., field, duration, and OFF and ON times), the resulting response surface occupies a four-dimensional volume. This situation also exists for the combination of field-duration-duty-ON time. Because the combination of field-duration-duty-ON time provides a natural comparison of the effects of pulse width on rectal ∆Tcorr at constant power and because a rigorous comparison of the two analyses produced substantially similar results, the results of modeling the combination of field-duration-duty-ON time are presented. Figure 5 shows a representative series of contour plots generated from the model equation showing rectal ∆Tcorr for various combinations of duration and duty at 950 Oe with varying ON times (Fig. 5A-C). Shading, a measure of the SE, within the plots indicates a relatively high degree of uncertainty, with ±3°C for darkest shading. Thus, quantitative predictions obtained from the model are limited.
Nevertheless, the trend of decreasing rectal ∆Tcorr with increasing ON (and therefore OFF) at constant duty is apparent. Particularly interesting is the change of rectal ∆Tcorr isotherms, which are generally increasing with increasing duration at constant duty and ON times of 15 and 60 seconds (Fig. 5A and B, respectively), and then tend to remain constant for increasing duration at a given duty (Fig. 5C).
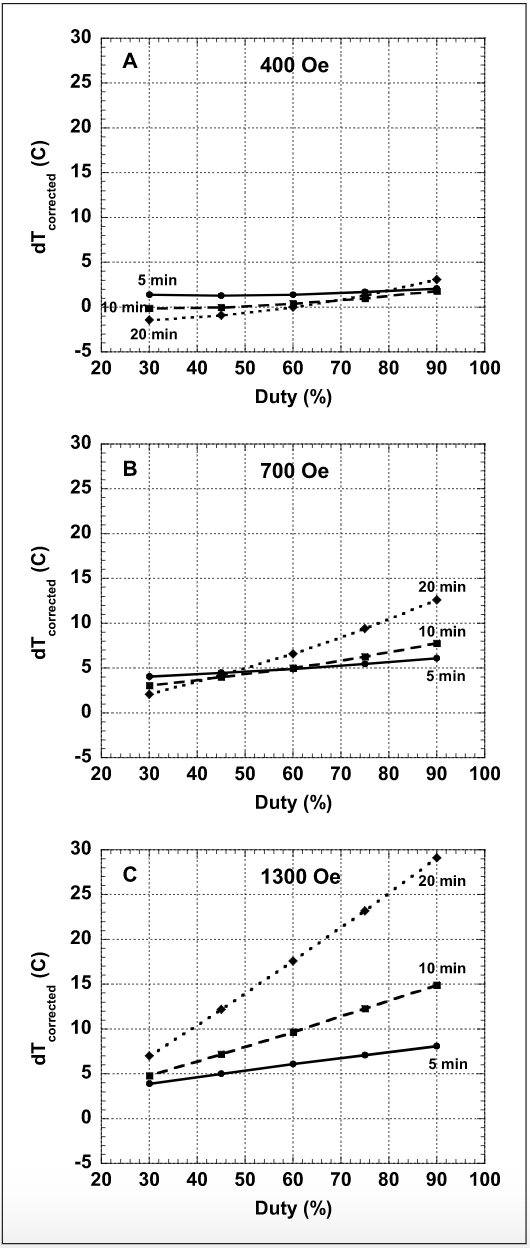
The trend of increasingly effective tissue temperature regulation for increased ON/OFF time for specific amplitude and duty combinations is further illustrated in Fig. 6A to C where duty is constant at 50%. At low fields (400 Oe; Fig. 6A), there is insufficient power deposition to create a net increase of rectal ∆Tcorr, regardless of ON time and duration of exposure. Indeed, a net loss of heat occurs with increased duration of exposure and ON time, as seen in Fig. 4. However, at 950 Oe (Fig. 6B), the combination of short ON time (5 seconds) and long duration (20 minutes) deposits a sufficient eddy current heating to approach a toxic threshold of ∆Tcorr = 13°C. However, further increasing the ON time to 90 seconds results in an apparently more effective in vivo temperature regulation yielding a net increase of ∆Tcorr 3°C that remains unchanged even at a 20-minute exposure, suggesting an equilibrium of heat input and output has been established. For 1,300 Oe (Fig. 6C), this equilibrium occurs at ∆Tcorr 5°C and 180 seconds ON time. On the other hand, a 5-second ON time produces a net increase of rectal ∆Tcorr with increasing duration of exposure, such that at 20 minutes, the toxic threshold will probably be exceeded.
Discussion
AMF can be used to heat magnetic nanoparticles localized in tissue (e.g., cancer) by specific antibodies. To effectively heat the cancer, it is advantageous to subject the particles to high-amplitude AMF of sufficient duration. This unique treatment approach takes advantage of the particle SAR, measured in W/g material, to focus heat on the cancer cells to initiate thermoablative therapy. Provided the AMF can be applied in a manner that limits net tissue temperature increase, healthy tissues are not subjected to extreme heat and are spared.
One way to reduce eddy current heating is to limit the area of exposure to high-amplitude fields. An induction coil was designed and manufactured to take advantage of mouse geometry and confine the area of high-amplitude AMF to a 1-cm-wide region inside the coil. The field distribution within the coil was inhomogeneous, thereby minimizing exposure to high-amplitude AMF in unintended areas. Placing magnetic flux controlling material on the coil achieved further reductions of flux in these areas.
No adverse events occurred for mice exposed to continuous AMF (100% duty) for amplitudes of 700 Oe and all durations (Table 1). Adverse events occurred for mice exposed for 20 minutes to continuous AMF at 100% duty and amplitudes of >700 Oe, with rectal temperatures approaching or exceeding 42°C. Whereas the sample size of this study was insufficient to determine the precise threshold for adverse events associated with AMF exposure, our observations are consistent with those made by others that core temperatures ≥42°C are potentially lethal (13).
More information was obtained from the data by fitting an empirical model to the ∆Tcorr data when field, duration, and duty were explicitly modeled. Assuming that a ∆Tcorr = 13°C is lethal, then a 20-minute exposure to field amplitudes as low as ~630 Oe and 100% duty can be lethal (cf. Fig. 3). This value is consistent but lower than might be predicted from inspection of Table 1.
Pulsing the AMF reduces heating by reducing total power deposited due to eddy current production and providing opportunity for heat dissipation during the OFF time. A linear relationship between ∆Tcorr and duty for a given field amplitude and duration is expected, because total power deposition due to eddy current production varies linearly with duty. Contour plots of field and duty at 20-minute duration (Fig. 3A-D) show that ∆Tcorr for spine, flank, skin, and rectum increases as duty is increased for a given field amplitude. The distance between successive ∆Tcorr isotherms seems constant for constant field, except at the lowest amplitudes and duties where some deviation from linearity appears. This apparent deviation is not significant, because in these regions the uncertainties are ±1°C, comparable with the predicted ∆Tcorr.
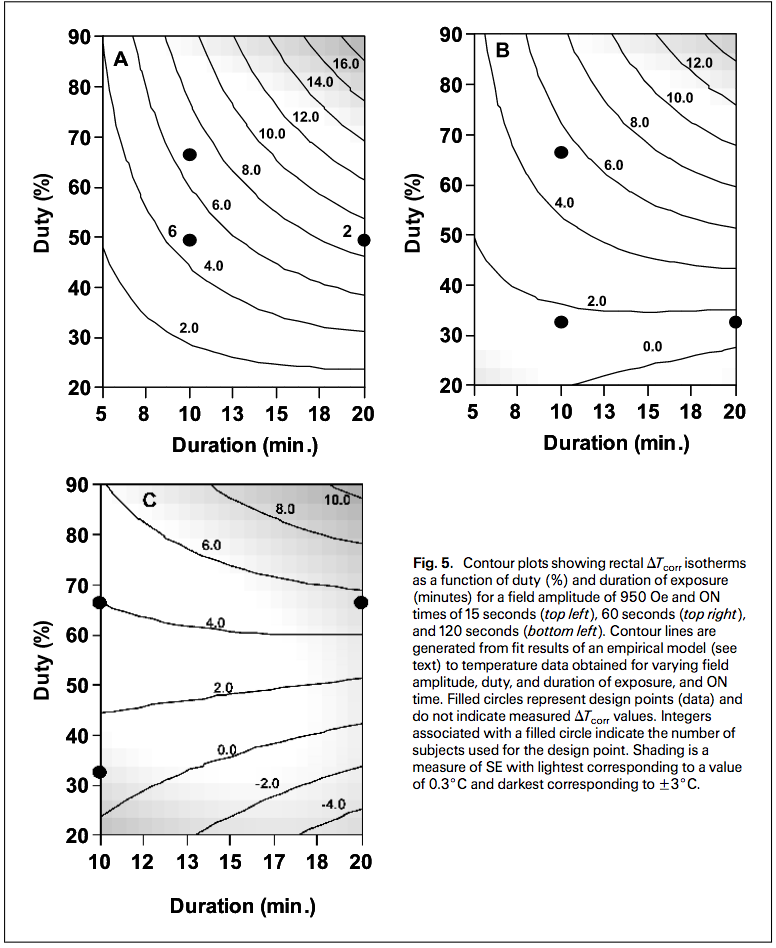
The value of ∆Tcorr for the rectum is lower than the corresponding temperatures for the spine, flank, and skin, particularly at high amplitude and duty (cf. Fig. 3A-D and Table 2). In general, eddy current production is greatest in areas with large radius and zero at the electrical center (i.e., r = 0; cf. Eq. A). The temperature probe placed on the skin is at maximum r for the mouse, whereas the temperature probe placed in the rectum is near the electrical center (i.e., r ≈ 0). The temperature probe placed on the skin provides a direct measure of eddy current heating during an ON cycle, with concomitant heat loss during an OFF cycle (Fig. 2A). Hence, temperature oscillations due to pulsing are pronounced, particularly with long ON/OFF cycles.
On the other hand, the temperature probe placed in the rectum does not measure eddy current heating directly but rather the net heat balance resulting from heating of the surrounding tissue during exposure to AMF, thermoregulatory response to eddy currents, heat loss to the environment (coil), and heat produced by metabolic processes. Consequently, temperature oscillations seem damped (Fig. 2B) when compared against skin measurements (Fig. 2A). Because mortality is associated more directly with core temperature (i.e., rectal; refs. 12, 13), maintaining a rectal temperature within safe limits has significant implications for the safe application of AMF.
Eddy current heating is most affected by duty at the highest amplitudes (~1,300 Oe; Fig. 4). That duty has such an effect upon ∆Tcorr for AMF exposure is not surprising as this is directly related to average power or dose, even for mice, which are known to have poor thermoregulatory systems (12), compared with humans. In addition to decreasing the total eddy current heating in the mouse, decreasing duty also provides time for heat dissipation during OFF cycles.
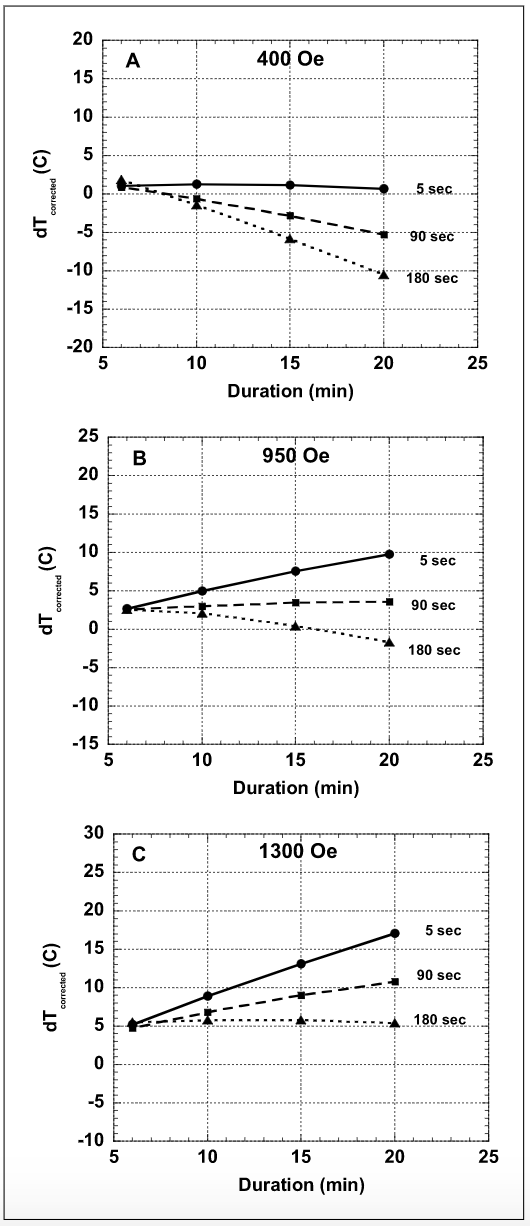
The effect of ON/OFF pulse variation for a given duty and amplitude was observed directly as illustrated in temperature plots shown in Fig. 2. Increasing ON time from 5 to 15 seconds results in an increase of eddy current heating and a net increase in temperature. However, this trend is reversed for very long ON/OFF cycles (»15 seconds ON and »30 seconds OFF), provided one ON cycle does not produce an acute level of eddy current heating (cf. Tables 1 and 2). Within this constraint, very long ON/OFF cycles can produce a net rectal temperature (Fig. 2B) that is substantially lower than that produced by short ON/OFF AMF exposure even after a 20-minute duration (Figs. 4 and 5). This is an intriguing observation.
Analysis of the data taking into account pulse width provides further evidence of the complex interaction of AMF power deposition (or tissue SAR) through eddy current production and resulting changes in tissue temperature. Contour plots showing rectal ∆Tcorr isotherms as a function of duty and duration of exposure for 950 Oe that result from an analysis of temperature response to the factors field amplitude-duration-duty-ON time is shown in Fig. 5A to C, where ON time is varied from 15 seconds (Fig. 5A) to 120 seconds (Fig. 5B). As ON time increases, the temperature in the rectum decreases, particularly at high duty and long duration of exposure, as does the slope and curvature of the individual isotherms. This qualitative change in temperature response with ON time suggests a profound shift in thermoregulatory response to AMF eddy current production as ON time is increased when field amplitude and duty, or total AMF ‘‘dose’’, are held constant.
This effect is further illustrated in Fig. 6A to C, which show a comparison of predicted rectal ∆Tcorr for a duty of 50% and ON times of 5, 90, and 180 seconds for field amplitudes of 400 Oe (Fig. 6A), 950 Oe (Fig. 6B), and 1,300 Oe (Fig. 6C). Note that for each field amplitude the average AMF ‘‘dose’’ (or tissue SAR) is constant, because it is defined by field amplitude, frequency, radius of exposure (cf. Eq. A), and duty. Conversely, the physiologic temperature response to this dose is complex and can vary dramatically depending upon the algorithm used to apply the AMF in vivo. Such a complex relationship of power deposition, tissue SAR, and tissue temperature response has been reported in both preclinical and clinical trials using microwave antenna arrays (12, 14 – 18) and ultrasound (19) for the treatment of cancer. Although the clinical significance of these observations at the frequency chosen in this study is unknown, it provides an interesting line for further investigation.
Safe application of high-amplitude AMF sufficient to heat antibody-conjugated magnetic nanoparticles (bioprobes) localized in cancer tissue is possible provided the area of exposure and duty are controlled. The choice of duration and duty at amplitudes >700 Oe are integral to limit nonspecific heating. Results presented suggest that pulse width is also important for safety and can be maximized, within limits, to apply a high thermal dose locally with nanoparticles to cancer cells. Under such conditions, heat delivered by bioprobes can potentially be modulated by suitable selection of AMF variables to enhance a therapeutic effect, whereas normal tissues can recover and dissipate heat produced by eddy currents during OFF times.
Acknowledgements
We thank Dr. Douglas Gwost and Carmen Traxler for their contributions to the study design and Laird Meiers for mouse care and handling.
References
[1] Overgaard J. History and heritage: an introduction. In: Overgaard J, editor. Hyperthermic oncology. Vol. 2. London: Taylor and Francis; 1985. p. 8-9.
[2] Streffer C, van Beuningen D. The biological basis for tumor therapy by hyperthermia and radiation. In: Streffer J, editor. Hyperthermia and the therapy of malignant tumors. Berlin: Springer; 1987. p. 24-70.
[3] Zaffaroni N, Fliorentini G, De Giorgi U. Hyperthermia and hypoxia: new developments in anticancer chemotherapy. Eur J Surg Oncol 2001; 27:340-2.
[4] Hildebrandt B, Wust P, Ahlers O, et al. The cellular and molecular basis of hyperthermia. Crit Rev Oncol Hematol 2002; 43:33-56.
[5] Moroz P, Jones SK, Gray BN. Tumor response to arterial embolization hyperthermia and direct injection hyperthemia in a rabbit liver tumor model. J Surg Oncol 2002; 80:149-56.
[6] Moroz P, Jones SK, Gray BN. Magnetically mediated hyperthermia: current status and future directions. Int J Hyperthermia 2002;18:267-84.
[7] Jeffrey S, Birdwell R, Ikeda D, et al. Radiofrequency ablation of breast cancer: first report of an emerging technology. Arch Surg 1999; 134:1064-2008.
[8] Izzo F, Barnett CC, Curley SA. Radiofrequency ablation of primary and metastatic malignant liver tumors. Adv Surg 2001; 35:225-50.
[9] Gordon RT, Hines JR, Gordon D. Intracellular hyperthermia: a biophysical approach to cancer treatment via intracellular temperature and biophysical alteration. Med Hypotheses 1979; 5:83-102.
[10] Jordan A, Wust P, Scholz R, Faehling H, Krause J, Felix R. Magnetic fluid hyperthermia (MFH). In: Hafeli U, et al, editors. Scientific and Clinical Appl Magn Carriers. New York: Plenum Press; 1997. p. 569-95.
[11] Jordan A, Scholz R, Wust P, Fahling H, Felix R. Magnetic fluid hyperthermia: cancer treatment with AC magnetic field induced excitation of biocompatible superparamagnetic nanoparticles. J Mag Mag Mat 1999; 201:413-9.
[12] Adair ER, Black DR. Thermoregulatory responses to RF energy absorption. Bioelectromagnetics 2003; 6:S17-38.
[13] Dewhirst MW, Viglianti BL, Lora-Michiels M, Hanson M, Hoopes PJ. Basic principles of thermal dosimetry and thermal thresholds for tissue damage from hyperthermia. Int J Hyperthermia 2003; 19:267-94.
[14] Mechling JA, Strohbehn JW, Ryan TP. Three-dimensional theoretical temperature distributions produced by 915 MHz dipole antenna arrays with varying insertion depths in muscle tissue. Int J Radiat Oncol Biol Phys 1992; 22:131-8.
[15] Ryan TP. Comparison of six microwave antennas for hyperthermia treatment of cancer: Sar results for single antennas and arrays. Int J Radiat Oncol Biol Phys 1991; 21:403-13.
[16] Ryan TP, Mechling JA, Strohbehn JW. Absorbed power depostion for various insertion depths for 915 MHz interstitial dipole antenna arrays: experiment versus theory. Int J Radiat Oncol Biol Phys 1990; 19:377-87.
[17] Trembly BS, Douple EB, Ryan TP, Hoopes PJ. Effect of phase modulation on the temperature distribution of a microwave hyperthermia antenna array in vivo. Int J Hyperthermia 1994; 10:691-705.
[18] Ryan TP, Hoopes PJ, Taylor JH, et al. Experimental brain hyperthermia: techniques for heat delivery and thermometry. Int J Radiat Oncol Biol Phys 1991; 20:739-50.
[19] Ryan TP, Hartrov A, Colacchio TA, Coughlin CT, Stafford JH, Hoopes PJ. Analysis and testing of a concentric ring applicator for ultrasound hyperthermia with clinical results. Int J Hyperthermia 1991; 7:587-603.
If you have more questions, require service or just need general information, we are here to help.
Our knowledgeable Customer Service team is available during business hours to answer your questions in regard to Fluxtrol product, pricing, ordering and other information. If you have technical questions about induction heating, material properties, our engineering and educational services, please contact our experts by phone, e-mail or mail.
Fluxtrol Inc.
1388 Atlantic Boulevard,
Auburn Hills, MI 48326
Telephone: +1-800-224-5522
Outside USA: 1-248-393-2000
FAX: +1-248-393-0277